We’ve never understood how hunger works. That might be about to change.
Scientists have spent decades trying to unravel the intricate mysteries of the human appetite. Are they on the verge of finally determining how this basic drive functions?
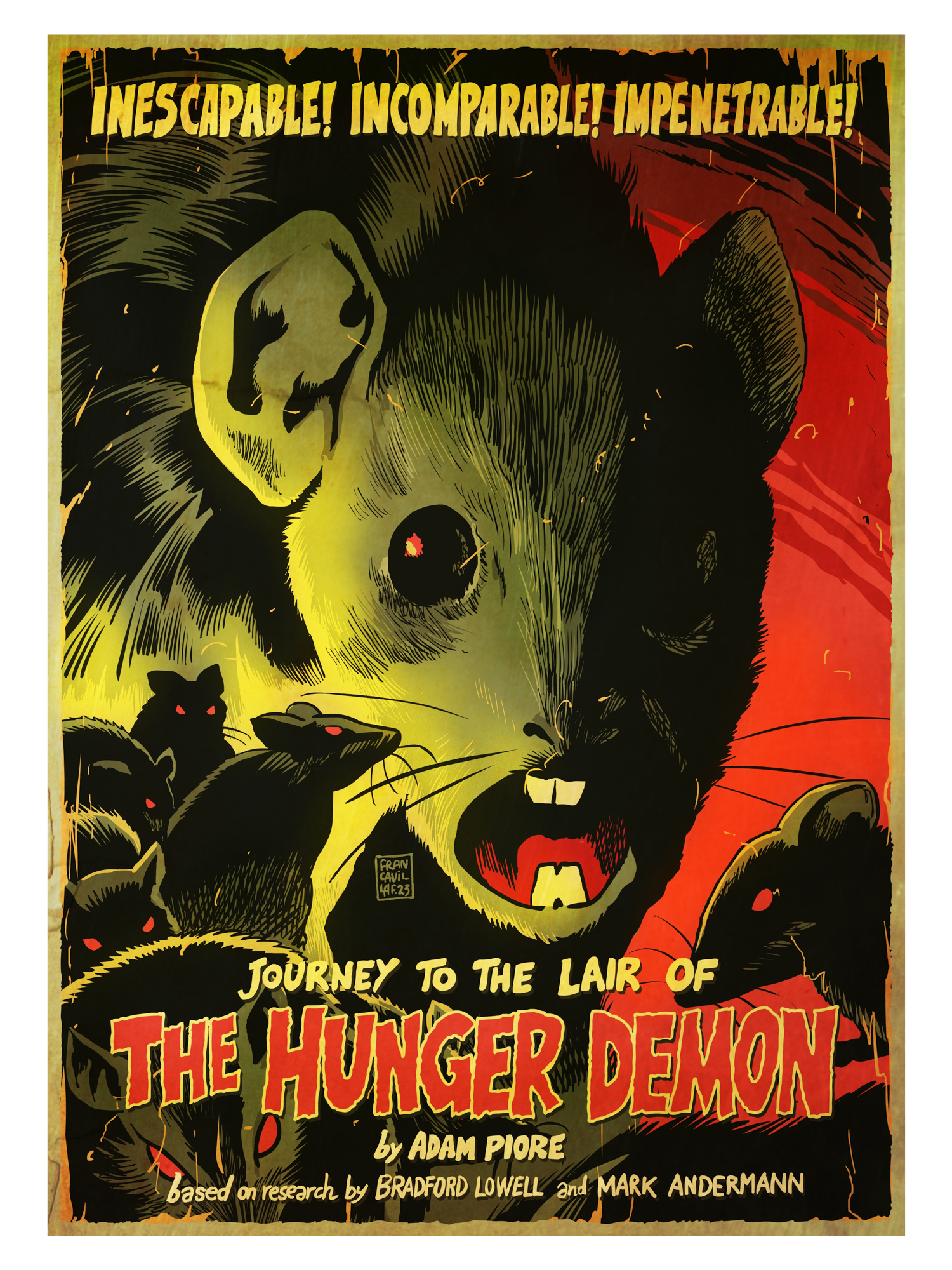
You haven’t seen hungry until you’ve seen Brad Lowell’s mice.
A few years ago, Lowell—a Harvard University neuroscientist—and a postdoc, Mike Krashes, figured out how to turn up the volume on the drive for food as high as it can go. They did it by stimulating a bundle of neurons in the hypothalamus, an area of the brain thought to play a key role in regulating our basic needs.
A video captures what happened next. Initially, the scene is calm as a camera pans slowly along a series of plastic cages, each occupied by a docile, well-fed mouse, reclining on a bed of wood chips. None of the eight mice shown are interested in the food pellets arrayed above them on the other side of a triangular metal grate that drops down from the ceiling. Which is not surprising, since each mouse has just consumed the rodent equivalent of a Thanksgiving dinner.
But as the seconds displayed on a timer at the bottom of the screen tick away, half the mice begin to stir—the first evidence that a chemical agent designed to turn on specific neurons associated with appetite is reaching its targets.
Soon, the mice seem possessed. Some stand on their hind legs, thrusting their noses through the grates above them at the inaccessible pellets. Others climb the walls, hang from the bars of the grate, or dig frantically through the wood chips.
“It looks like they’re losing their minds,” Lowell says.
Lowell, who is one of the world’s leading experts on the circuits in the brain that control hunger, satiety, and weight regulation, sometimes references this video to make a point: When you’re starving, hunger is like a demon. It awakens in the most ancient and primitive parts of the brain and then commandeers other neural machinery to do its bidding until it gets what it wants.
“Sure, we managed to have the brain say ‘Go eat,’” Lowell says. “But that’s not really an explanation. How does that actually work?”
What might begin as a small sensation quickly spirals. Intrusive thoughts pulled from our memory centers burst into our consciousness. Images of meatball sandwiches. The smell of bread. The imagined taste of a cork-like food pellet. The motivational and emotional areas of our brain infuse the need to eat with a nonverbal imperative that feels so powerful it eclipses all else. Our prefrontal cortex kicks into gear, considering how we might obtain food. (If we are in a dangerous situation like a war zone, we weigh how much danger we are willing to risk to get it.) Then we mobilize our sensory and motor areas. We steal a chicken, attempt to spear a fish in a pond, raid the work refrigerator, or hurl our body against a metal grate, hoping to get a taste of a food pellet.
So by exciting the hunger neurons in those mice, Lowell catalyzed a storm of neural activity that spread to the cerebral cortex and other higher-order processing centers, leading directly to a chain of complex goal-directed behaviors (ineffective though they turned out to be).
It also drove home for Lowell just how much we still have to learn.
“Sure, we managed to have the brain say ‘Go eat,’” he says. “But that’s not really an explanation. How does that actually work?”
To answer that question, Lowell has teamed up with Mark Andermann, a neuroscientist who studies how motivation shapes perception (and who also happens to occupy the office next to his at Boston’s Beth Israel Deaconess Medical Center). Together they are following known parts of the neural hunger circuits into uncharted parts of the brain, in some cases activating one neuron at a time to methodically trace new connections through areas so primitive that we share them with lizards.
Their work could have important implications for public health. More than 1.9 billion adults worldwide are overweight and more than 650 million are obese, a condition correlated with a wide range of chronic health conditions, including diabetes, fatty liver disease, heart disease, and some types of cancer. Understanding the circuits involved could shed new light on the factors that have caused those numbers to skyrocket in recent years.
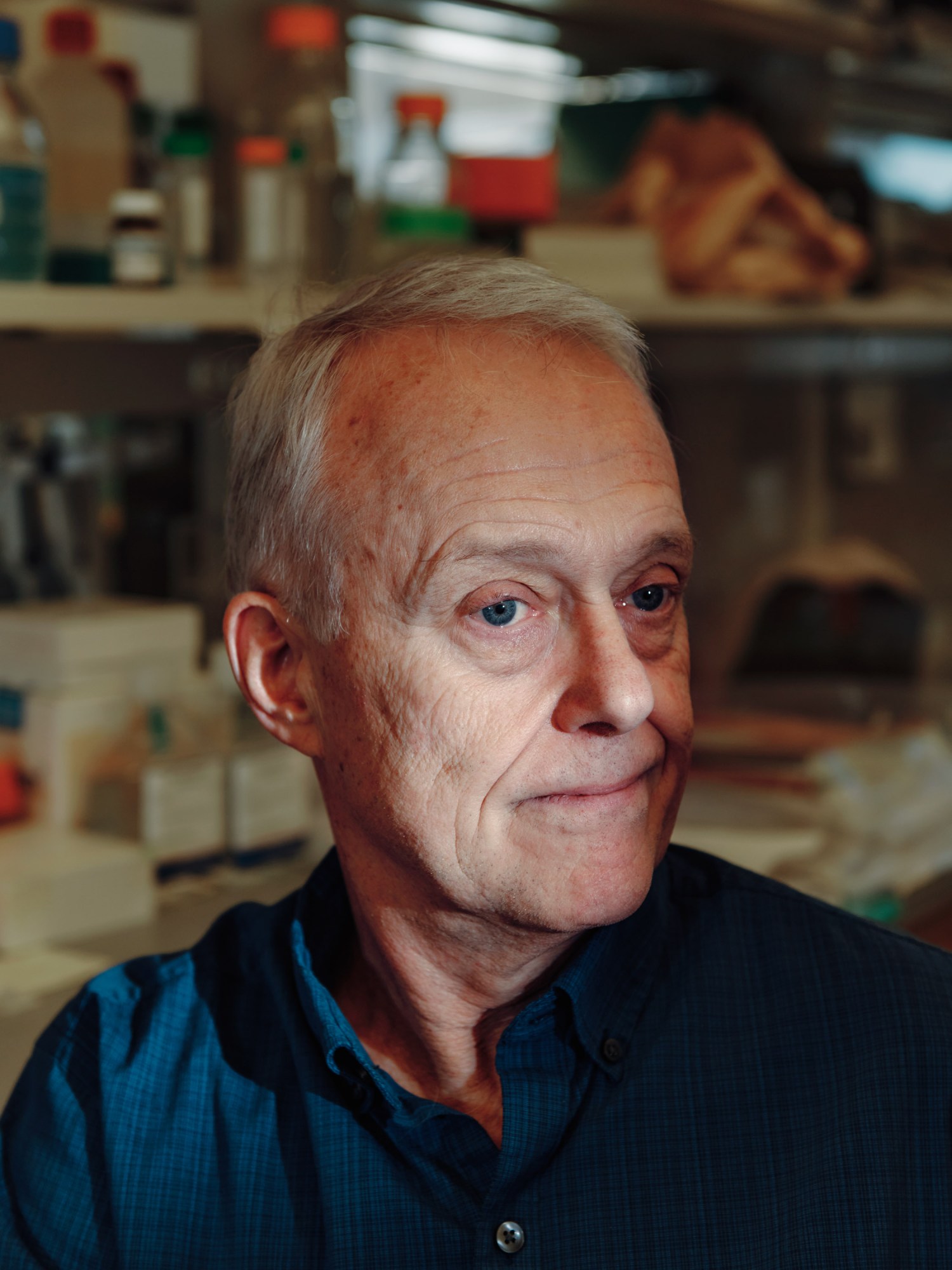
And it could also help solve the mystery behind a new class of weight-loss drugs known as GLP-1 agonists. Many in the field of public health are billing these drugs, which include Wegovy and Ozempic, as transformative, providing the first effective method of combating obesity, and allowing some individuals to lose more than 15% of their body weight. They’ve also become something of a cultural phenomenon; in the last three months of 2022, US health-care providers wrote more than 9 million prescriptions for the drugs. Yet no one can explain precisely how and why they work. Part of the reason is that scientists still haven’t decoded the complex neural machinery involved in the control of appetite.
“The drugs are producing the good effects, the satiety effects, through some aspect of this larger system,” says Lowell, who has watched their emergence with surprise and genuine fascination. “One of the most important components in figuring out how they work is to define what the system is. And that is what we are doing.”
But the ultimate goal for Lowell and Andermann is far loftier than simply reverse-engineering the way hunger works. The scientists are searching for the elusive bundle of neurons that allow our instinctual urge to eat to commandeer higher-order brain structures involved in human motivation, decision-making, memory, conscious thought, and action. They believe identifying these neurons will make it possible to study how a simple basic impulse—in this case, a signal from the body that energy stores are beginning to run low and need to be replenished—propagates through the brain to dominate our conscious experience and turn into something far more complex: a series of complicated, often well-thought-out actions designed to get food.
This quest has so consumed Lowell in recent years that his graduate students have coined a term for the elusive bundle of brain cells he is seeking: “Holy Grail” neurons.
It might sound like a tired scientific trope. But for the understated Lowell, the term is perfectly apt: what he’s seeking gets at the very heart of human will. Finding it would be the culmination of decades of work, and something he never imagined would become possible in his lifetime.
The hunger mystery
Brad Lowell likes to joke that he is the token local at Beth Israel Deaconess Medical Center. Born in the hospital next door to where he now conducts research, he grew up 25 miles north in the town of Boxford and attended the University of Massachusetts, Amherst, a couple of hours’ drive away.
Soon after arriving at UMass as an undergrad in the late 1970s, he was accepted into the physiological psychology lab of Richard Gold, a pioneering neuroscientist who was working to identify neural structures involved in regulating appetite.
Gold’s focus was the hypothalamus—a primitive structure deep in the brain that hasn’t changed much through evolution. It is thought to be responsible for keeping the body in “homeostasis” by monitoring and balancing important functions like body temperature, blood pressure, our need for food and water, and other basic drives.
Gold suspected that the paraventricular hypothalamic nucleus (PVH), a tiny patch of roughly 50,000 neurons in the hypothalamus, played a role in controlling appetite. By today’s standards, the tools to study it back then were “stone age”—Lowell says he used a “retracting wire knife” to sever bundles of neuronal projections that emanated from the PVH and connected to neurons outside it—but they were effective. When the anesthetized rodents Lowell had operated on woke up, they were crazed with hunger, and they quickly became obese.
The experience made a lasting impression. Lowell, then an athletic 19-year-old soccer aficionado, had always assumed that anyone who was overweight was just “lazy.” The experiment suggested there was likely far more to it than that. It also convinced Lowell to become a scientist.
But further research into how precisely the brain worked to control hunger and satiety had reached something of an impasse.
“Gold and a few other labs put the PVH on the map as a site required to restrain what you eat,” Lowell explains. “But they didn’t have the tools to look any further.”
Figuring out which of the 50,000 neurons in the PVH were actually important to appetite, the ones that could essentially mute the hunger switch, was a challenge that seemed insurmountable—akin to, as Lowell puts it, trying to untangle a “huge bowl of spaghetti.”
“How do you differentiate one strand of spaghetti from another? These being neurons, right?” he asks. “There’s no way. They all look the same.”
When Lowell opened his own lab at Beth Israel Deaconess Medical Center in the early 1990s, after earning an MD and PhD at Boston University, he studied metabolism in tissues like muscle, organs, and fat that were connected to the brain through the peripheral nervous system. But his undergrad experience in Gold’s lab nagged at him.
“The brain is the Lord of the Rings,” Lowell says. “It’s the one ring that rules them all. And it was not that interesting to study these other things with the master player up there.”
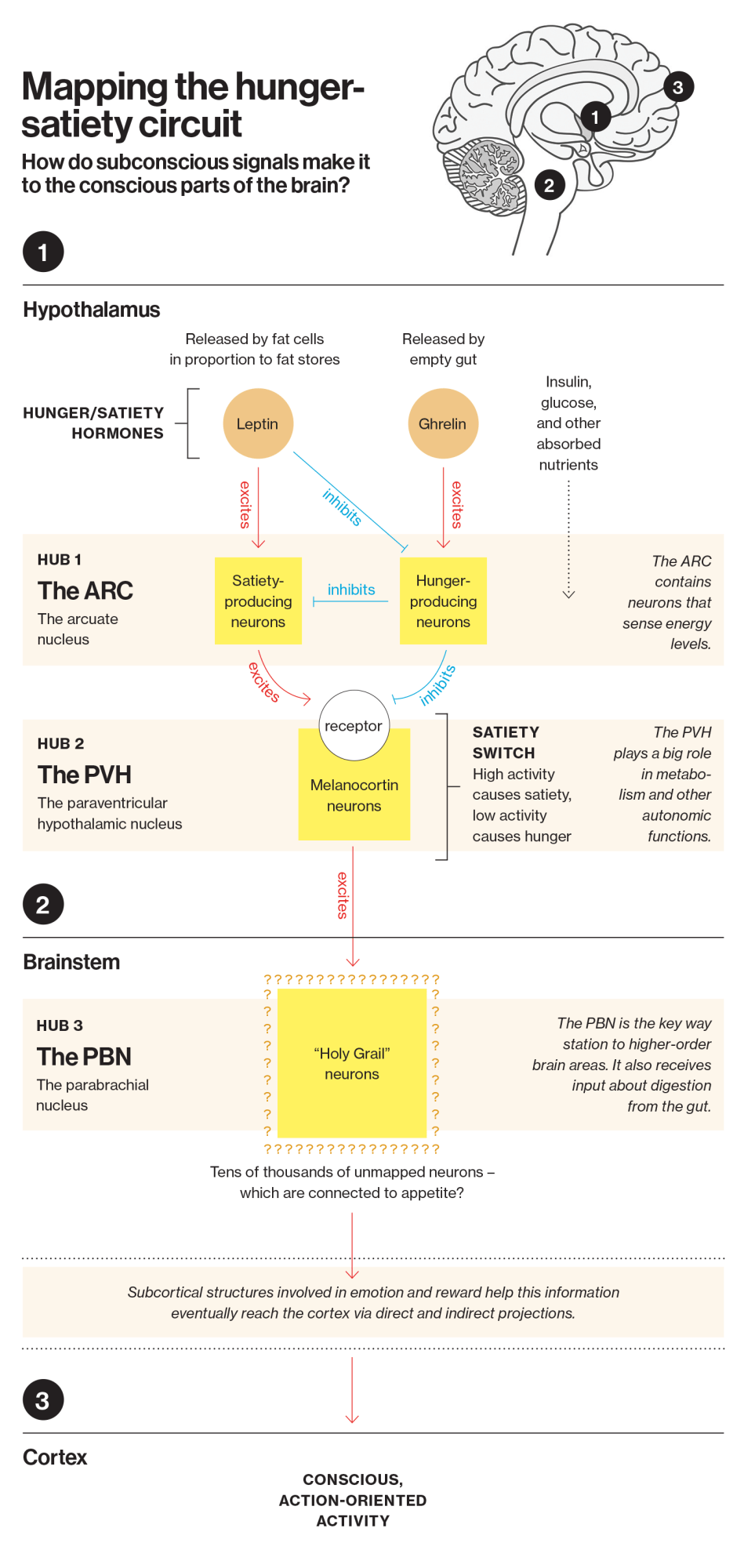
The entry point
Early in his career, Lowell envied his colleagues who studied vision. For decades, neuroscientists had been able to trace the neural circuits involved in that function by shining light into the eyes of mice, identifying which neurons lit up, and then following them to map out the relevant brain circuits. Lowell and his peers who were interested in hunger had never had a similar entry point.
That changed in 1994, when Jeffrey Friedman, a researcher at Rockefeller University, gave Lowell and others a way to identify the first important neurons—or individual “strands of spaghetti”—involved in hunger regulation.
Back in 1949, scientists at the Jackson Laboratory in Bar Harbor, Maine, had bred mice with an unidentified genetic mutation that caused them to grow massively obese. They hypothesized that the obesity stemmed from the mice’s inability to produce a crucial protein involved in weight regulation.
Decades later, Friedman was the first to apply cutting-edge genetic technologies to clone the DNA sequences that were abnormal in the obese mice; he then confirmed that their obesity was caused by an inability to produce a key hormone released by fat cells, which the brain uses to track the body’s available energy stores. Friedman purified the hormone and named it leptin. He also identified the DNA sequence needed to make the leptin “receptor”—the specialized proteins that stick out of brain cells involved in appetite regulation like microscopic antennae, sensing whenever leptin is present and kicking off a chemical cascade that promotes a sense of satiety.
The discovery added further evidence to the idea that obesity was biologically determined, and more specifically to the concept of a “set point” when it comes to weight—a predetermined weight, fat mass, or other measurable physiological characteristic that the body will defend. Appetite is the means by which the body performs “error correction” and mobilizes to devote energy and attention to the task of restoring homeostasis.
A “cure” for obesity suddenly seemed within reach. The biotech firm Amgen licensed the rights to leptin for $20 million, hoping to develop a drug that could mimic its effects. But the drug it came up with had very little effect on most people with obesity, suggesting that leptin was only part of the story—a hypothesis that seemed to be confirmed when other labs discovered additional hormones and signals that seemed to be involved in hunger. Further experiments showed that many obese humans in fact had normal or high levels of leptin.
It stood to reason, then, that somewhere in the brain leptin was being combined with other signals related to available energy, and that this information would then have to be compared with a homeostatic “set point.”
This suggested a highly complex set of neurological circuits involved in hunger regulation. Understanding how this process worked would require a detailed wiring diagram that might explain how all the parts fit together. And while Friedman’s discoveries regarding leptin didn’t answer all the questions, they provided the entry point that Lowell and the rest of the field had been waiting for, allowing them to begin to draw such a map.
Following the path of leptin, scientists in other labs found the hormone’s first target, and therefore the first important way station in the hunger circuit: a specific patch of neurons known as the arcuate nucleus (ARC). Located at the base of the hypothalamus, the ARC, we now know, integrates information coming from other brain structures, as well as circulating nutrients and hormones like leptin and insulin. All of these inputs convey key information about the current state of the body, such as the level of existing energy stores and nutrient availability.
Determining how the ARC worked—and where it sent information after taking it in—was the next question facing the field. By then, Lowell had abandoned studies on peripheral systems and joined the hunt.
Switching hunger on and off
In 1997, the next part of the puzzle fell into place after Roger Cone, then a researcher at Oregon Health and Science University, discovered a key part of the switch that essentially turned hunger on and off.
He bred mice with a gene mutation that interferes with another class of key signaling proteins, called melanocortins. Mice with this mutation more closely resembled obese humans than did mice with leptin mutations: their obesity set in relatively late, and they had diabetes-causing levels of insulin and glucose. This particular mutation prevented key receptors from detecting melanocortin hormones, which in turn interfered with the feeling of satiety and caused mice to continue to eat. But when these melanocortin receptors were functioning normally, detecting the presence of the melanocortin hormones seemed to turn down appetite. In essence, Cone had found the brain’s “satiety switches.”
This discovery was critical in helping scientists determine how leptin worked its magic in the ARC, the first stop in the hunger circuit. It turned out that when leptin reached the ARC, it set off a biochemical chain reaction that caused more melanocortin hormones to be released, eventually activating these “satiety switches.”
But these satiety switches were not present just in the ARC; they were on neurons distributed throughout the hypothalamus, the hindbrain, and the forebrain, suggesting that one of these areas was the next key hub in the hunger circuit. So which one was it?
It still did not answer perhaps the most fascinating question of all: How did these signals eventually make it into the conscious parts of the brain?
Some of these switches were in the paraventricular hypothalamic nucleus—the brain area Lowell had studied in the lab of Richard Gold as an undergraduate. Since Lowell had seen with his own eyes that mice ate voraciously if you took it offline, he had long believed the PVH to be a stop in that circuit.
Now he had the tools to prove it. Over the years, Lowell had developed an expertise in cutting-edge genetic engineering techniques that allowed him to target and delete specific genes and create new strains of “knockout” mice—meaning specific genes had been knocked out in an embryo, causing a mouse to be born without a functional copy.
In 2005, Lowell and a colleague, Joel Elmquist, engineered mice to carry a genetic sequence that prevented them from making functional copies of satiety switches anywhere in the brain. As expected, the mice grew obese.
Lowell and Elmquist then created pairs of microscopic molecular scissors. Using genes unique to neurons in the PVH as a homing beacon, they programmed these scissors to seek out only DNA associated with PVH neurons and snip away the small sequence that prevented the development of functional satiety switches in that part of the brain. In other words, they “fixed” the satiety switches in the PVH, while they remained disabled in the rest of the brain. If the PVH was where the magic happened, restoring the satiety switches there would fix the problem of obesity.
Indeed, Lowell’s knockout mice were effectively “cured” of obesity—confirming his hypothesis. He had proved that the PVH was the next key relay point in the hunger-satiety circuit.
For Lowell, confirming the PVH’s place in the circuit was huge‚ but it still did not answer perhaps the most fascinating question of all: How did these signals eventually make it into the conscious parts of the brain, the parts that could make an animal take action to get food? How did hunger, in other words, manage to commandeer the neural machinery of those crazed mice? How do intrusive thoughts of a meatball sandwich compel someone to put on shoes and a coat and track one down?
To find out, Lowell needed to determine where the signals in the PVH led, in the hopes that if he continued to follow the string it would lead him to the gateway to higher-order brain structures. This was complicated by the fact that neurons in the PVH sent signals to a number of different areas, including the brain stem, regions that affect thyroid function, and others.
Lowell was stymied. “We could knock out these genes and then measure how much food the mice ate or measure how fat they got, but we couldn’t go much further,” he says.
A magic “remote control”
In the summer of 2009, four years after the PVH discovery, Lowell was visiting Colgate in upstate New York with his high-school-age son. Lying on the grass outside the administrative building while his son did an interview, he flipped open the latest issue of the scientific journal Neuron. An article detailed a new laboratory tool developed by Bryan Roth at the University of North Carolina, Chapel Hill: a “chemical-genetic remote control” that could be used to turn specific neurons on and off in mice. Lowell recognized instantly it was the breakthrough he had been waiting for his entire career.
Instead of just knocking out populations of neurons permanently in mice, Lowell could instead create new strains of mice that were bred to have this genetic “remote control” switch, allowing him to turn distinct populations of neurons on and off simply by administering a chemical agent. (A separate technique known as optogenetics also allows him to do this by beaming a specific wavelength of light into the brain through a fiber-optic cable.) He could then observe the behavioral effect of turning specific neurons on and off in real time.
“Suddenly I was able to do things that when I was an undergraduate I never dreamed I’d be able to do,” he says.
In 2014, Lowell used the remote-control tool to methodically turn each bundle of neurons leading out of the PVH on and off, to see which ones produced satiety. Once he identified the neurons that affected satiety, he followed them out of the hypothalamus. It led him to an area in the brain stem called the parabrachial nucleus (PBN)—the third key hub involved in the hunger-satiety circuit.
It was a scientific watershed. Lowell had finally arrived at an area of the brain with direct connections to higher-order brain structures affecting all aspects of our conscious experience, including areas involved in motivation, reward, emotion, processing sensory stimuli, memory, selective attention, and a wide array of other functions.
Somewhere in that area of the brain was the last way station, the “Holy Grail” neurons: those finally telling the rest of the brain to “go eat.”
Hunting for the Holy Grail
For the past eight years, Lowell and Andermann have been looking for the PBN neurons involved in hunger. It’s a painstaking hunt—the PBN contains hundreds of thousands of neurons. Lowell’s lab is tracing the hunger-satiety circuit forward out of the PBN while Andermann’s lab works backwards toward it from the insular cortex, an area associated with the conscious experience of bodily states like hunger. The goal is to meet in the middle.
If they can trace this circuit, then they will begin to examine how it is that a simple signal—a signal that we are hungry—works to recruit higher-order brain areas and focuses them on the completion of a task. They will have the opportunity to develop a model of how animals translate desire into action. Put simply, they might be able to characterize a complex action from beginning to end.
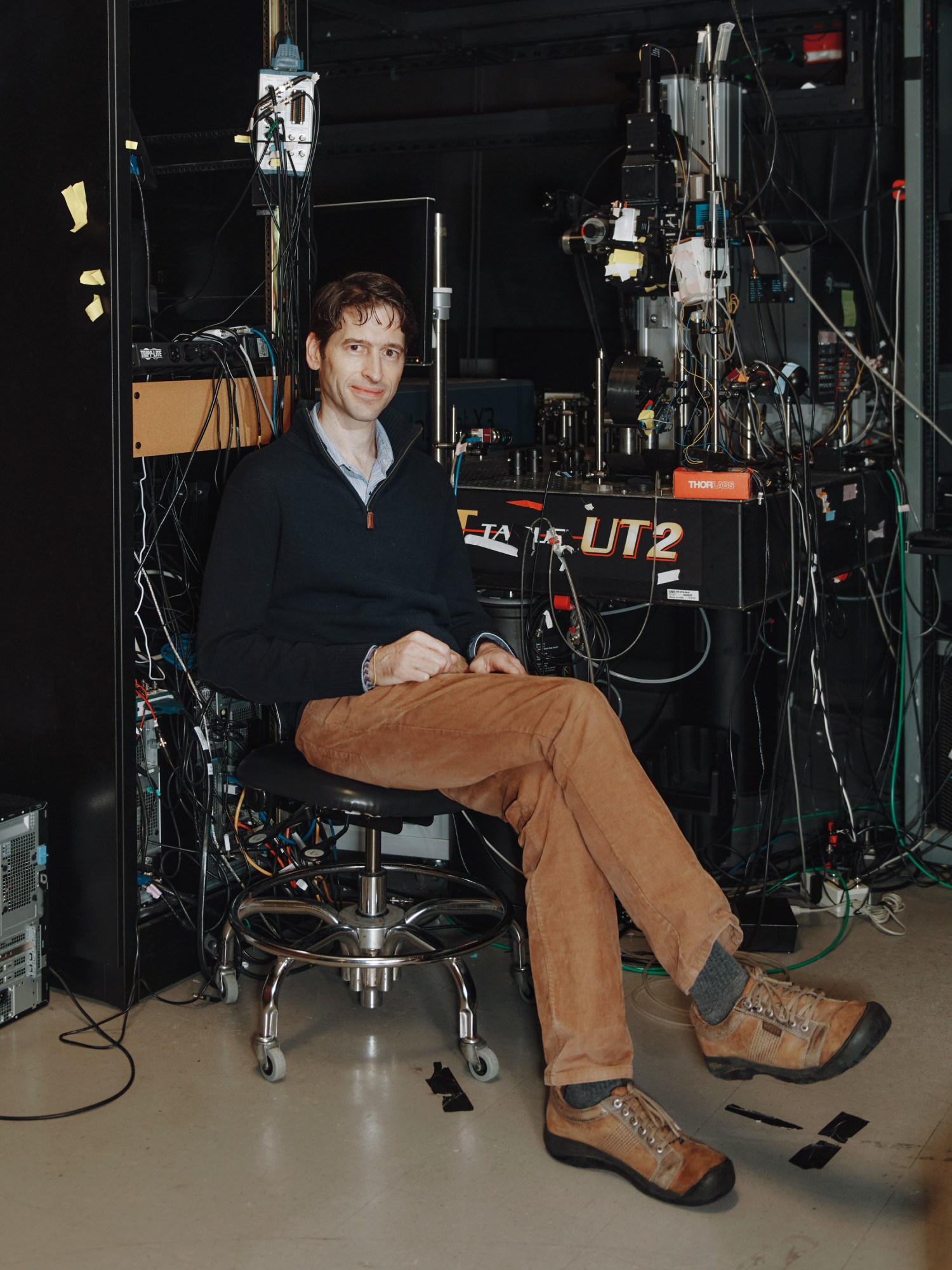
The sheer number of neurons in the PBN makes the task daunting. It’s made even more complicated by the fact that the PBN isn’t just involved in sending hunger signals to higher-order brain processing centers but is also the final stop for scores of other impulses and needs. It is a huge way station for all sorts of information, most of which has nothing to with hunger—like sexual arousal; the sensations associated with pain; the detection of heat and cold, itches and nausea; and signals associated with a wide array of autonomic functions, including respiration, blood pressure, and temperature regulation. Each one of these signals likely has its own set of dedicated, genetically distinct neurons in the PBN. Most of these neurons have never been identified or studied. And they all look identical.
At times, the researchers have had to trace the path of nerve impulses one neuron at a time—activating a neuron they know is part of the hunger-satiety circuit using the “remote control” technologies, and then watching to see which neurons light up in response. (The DNA of the mice he works with also contains sequences for fluorescent tracers that light up when certain neurons fire, and that light can be detected, using sophisticated optical sensing technology, through a window in the skull and then reproduced on a computer screen.) This has allowed Lowell and Andermann to reduce the number of candidate neurons he is considering from hundreds of thousands to about 10,000.
To further narrow down the possibilities, Lowell spent three years sorting these 10,000 neurons into different subtypes using their genetic signatures. He has identified 37 genetically distinct subtypes.
Now Lowell and Andermann are experimenting with subtype after subtype to see which ones are involved in the hunger circuit.
To do so, they are exposing live mice to different conditions and watching to see which neurons fire in response. They can see if a neuron fires when, for instance, the mice are shown pictures they’ve learned to associate with a tasty treat.
Once they identify neurons that are activated in the PBN by the food cue, they are using other experimental techniques to figure out which of the 37 distinct genetic profiles these neurons carry.
The process, which involves sacrificing the mice and dissecting their brain tissue, can be painstaking. But Lowell and Andermann insist they are closing in on their target. They hope that within the next five years they will have found the neurons they are looking for. From there, they can proceed into higher-order areas of the brain.
The recent development of the new class of weight-loss drugs—and the experiences reported by patients—tantalizingly illustrate how much power the circuits they are tracing can have on those areas. Not only is the physical experience of hunger absent—because the drugs seem to lower the body’s “set point”—but everything else that usually goes along with hunger seems to fade away. Patients report that they are no longer plagued by intrusive thoughts of food. (These reports parallel what Andermann and Lowell are seeing in the lab. Using their neural imaging techniques, the researchers can actually tell when mice are thinking about visual cues they have seen in the last minute or hour.)
It remains to be seen whether Lowell and Andermann’s work will actually resolve the intense debate in the field over how these drugs work, and what parts of the brain they act on. But the researchers hope that by decoding the circuit, their findings may inform the development of new generations of drugs that are even more effective and lack side effects such as nausea, vomiting, diarrhea, abdominal pain, and, in some cases, pancreatitis and changes in vision.
Though this would be newsworthy, it’s still not what excites Lowell the most. He remains most committed to the idea that his research could yield new insights into motivation, decision-making, and a wide array of other functions—into human will and survival. To illustrate why he is excited, he talks about a video he’s seen of a hungry squirrel navigating a “Mission Impossible” course to access food; the squirrel climbs up a pole, hurls itself through the air and lands on a windmill, and shimmies through a small opening in a plastic barrier while hanging upside-down from a clothesline.
“The squirrel isn’t operating on reflex,” he says. “It’s a totally novel environment. It has to use all of its higher processes to achieve that goal.” How does this very simple system manage to take over?
“That’s the big question,” he says. “We don’t know how any of that works, those higher processes.”
Now that he’s finally equipped with all the tools he needs to untangle the dizzyingly complex bowl of neural spaghetti, it may just be a matter of time before he finds out.
Adam Piore is a freelance journalist based in New York. He is the author of The Body Builders: Inside the Science of the Engineered Human, about how bioengineering is changing modern medicine.
Deep Dive
Biotechnology and health
The Biggest Questions: What is death?
New neuroscience is challenging our understanding of the dying process—bringing opportunities for the living.
I received the new gene-editing drug for sickle-cell disease. It changed my life.
As a patient enrolled in a clinical trial for Vertex’s new treatment, I was among the first to experience CRISPR’s transformative effects.
The lucky break behind the first CRISPR treatment
Gene editing for sickle-cell is here. This is how researchers knew what DNA to change.
Medical microrobots that can travel inside your body are (still) on their way
Microrobots released into the body could bust up clots, deliver cancer drugs, and even guide listless sperm to their target.
Stay connected
Get the latest updates from
MIT Technology Review
Discover special offers, top stories, upcoming events, and more.